Our IDQ Infrared Single Photon Detector may bring you some new insights.
Taking single-photon detection rates to new highs, with the ID281 parallel-pixel superconducting detectors.
Detecting single photons is hard. Not because they are strange wonders of quantum mechanics, as you might think, but because there are simply too many of them. One has a similar problem counting the number of drops of water in the ocean.
It’s a relatively simple matter1 to turn a single particle of light into a machine-readable signal. Photo- multiplier tubes (PMTs) and single-photon avalanche diodes (SPADs) are examples of detectors that rapidly turn a single photon into a large number of electrons, much like the clicks of a Geiger-Mueller tube detecting particles of ionising radiation. The state of the single-photon detection art, however, lies with superconducting nanowire detectors.
In this application note, we look at the challenge of high-speed single-photon counting, including the photon pile-up effect and a novel detection approach designed to circumvent this, allowing you to detect photons faster than ever before, above 200 MHz and beyond.
The state of the art: SNSPDs The present-day standard for single-photon detection is the superconducting nanowire single-photon detector (SNSPD). In this device, a single meandering line of superconducting material is held close to its superconducting threshold, where a single photon will create a localised but measurable resistance spike in the nanowire. This method provides high efficiency, precise timing and low noise, which allows SNSPDs a much wider range of photonic experiments than their SPAD counterparts: | 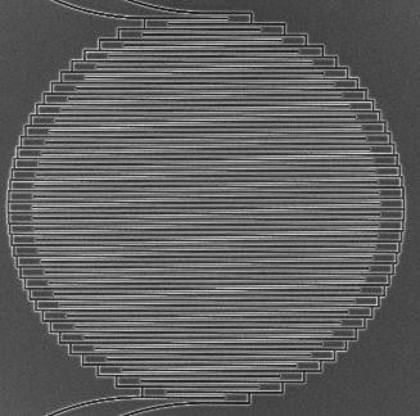
|
· Detection is near-ideally efficient. Close to 100% of individual photons are detected, compared to about 40% efficiency for the best SPADs.
· Timing is highly precise and robust. Pulses are repeatably measured within tens of picoseconds of each other, compared to the hundreds of picoseconds of the very best SPADs. This timing jitter is wavelength dependent: higher-energy (shorter-wavelength) photons will create a steeper response from the detector, and thus provide a greater level of timing precision. This timing jitter is usually the dominating factor in the time resolution of a detector system.
· Noise is ultra-low. Electrical noise in the detector is effectively zero, and by operating at cryogenic temperaturs, the number of black-body photons that may cause a false detection event can be less than one photon per second at visible wavelengths, but increases at higher wavelengths.
The Problem
Detectors can only count so many events before they begin to saturate. The maximum count rate a system can measure efficiently is dictated by the detector recovery time: each time a detector is triggered, it requires a short but finite time to reset its electrical configuration. Photons hitting the detector during this recovery time are not registered as detected events.
The result is known as the photon pile-up effect. When the incident flux of photons increases, the capacity to detect newly arriving photons decreases, and the overall system detection efficiency decreases (see Figure 1). The shorter the recovery time, the greater the maximum number of photons that can be detected well. For InGaAs SPADs, this recovery time is typically hundreds of nanoseconds, giving a maximum count rate of several MHz for the best systems. For SNSPDs, this time is shorter, typically recovering in a few tens of nanoseconds. As such, SNSPDs can have a maximum count rate of several tens of MHz before the detectors saturate.
Detecting fifty million-or-so photons per second is respectable, but can we do better?
Figure 1: Representation of the photon pile-up effect, demonstrated for detector inputs of approximately 2 MHz (top, green), 10 MHz (middle, red) and 50 MHz (bottom, blue). Detected photons are marked with a star, while missed photons that arrive at the detector too soon after a detection event are marked with an empty circle. The lower the single-photon rate, the greater the proportion of photons that are detected, and therefore the higher the system detection efficiency.
The Solution We can indeed do better. A neat solution to the photon pile-up effect is a parallel-pixel detector design, as recently demonstrated by Matthieu Perrenoud et al. [1], in a collaboration between IDQ and the group of Hugo Zbinden at the University of Geneva. Here, an array of superconducting detectors are connected electrically in parallel, to a single readout circuit (see Figure 2). While each individual pixel is still limited by a required recovery time, by distributing the signal to be measured across the array, the arriving photons are now much less likely to pile up at one detector, and considerably higher single-photon detection rates can be achieved. All this is still through a single-channel readout, which is a must- have for scaling this high-speed approach in an SNSPD system. If there was a separate readout channel for each pixel, the supporting electronics would quickly become very complex, and in the cryogenic environment of the SNSPD system, the added thermal load would degrade the stability and noise performance of the detector. This parallel pixel design, as such, is an elegantly scalable approach towards GHz-rate single- photon detection.
| 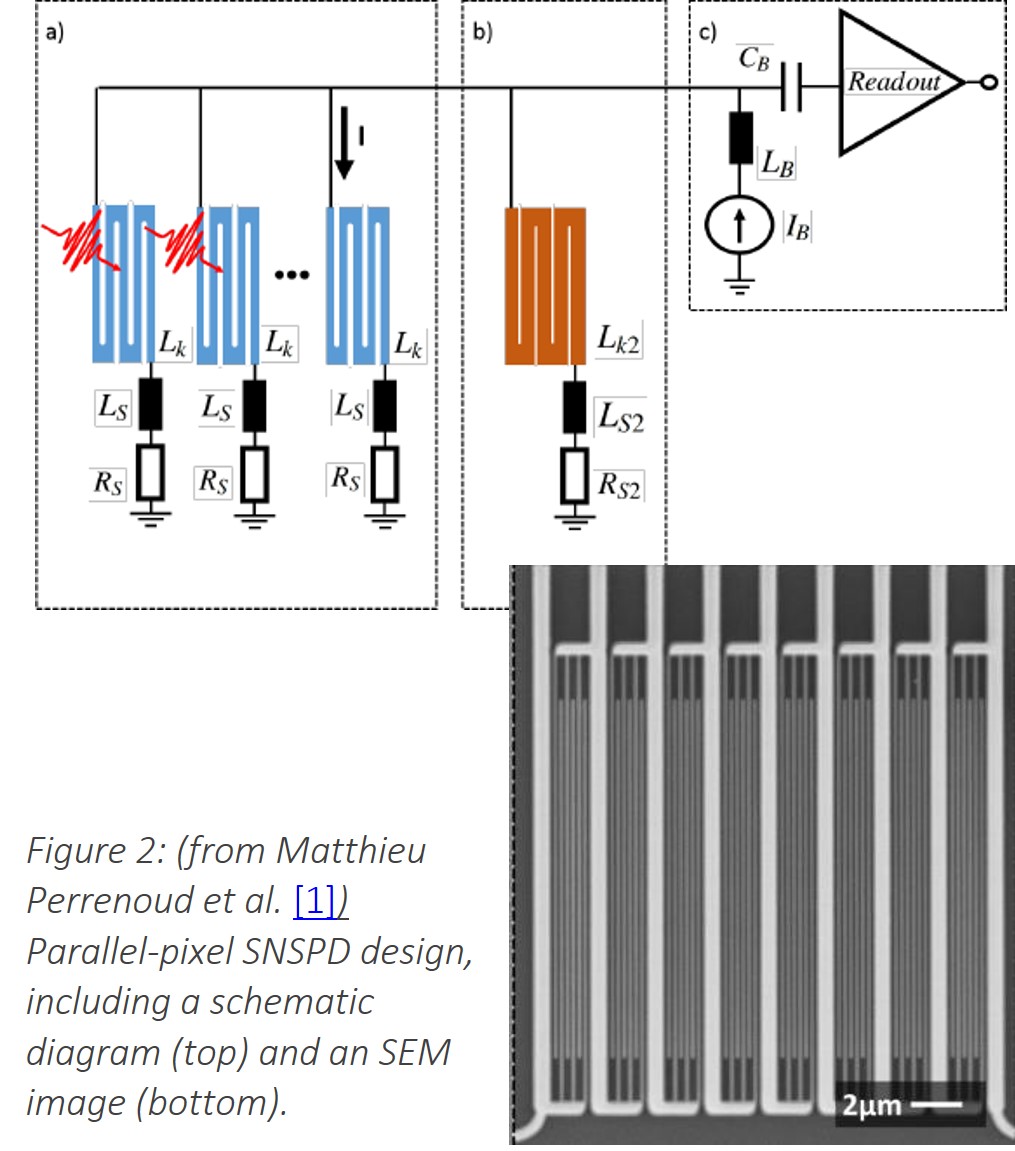
|
The Result
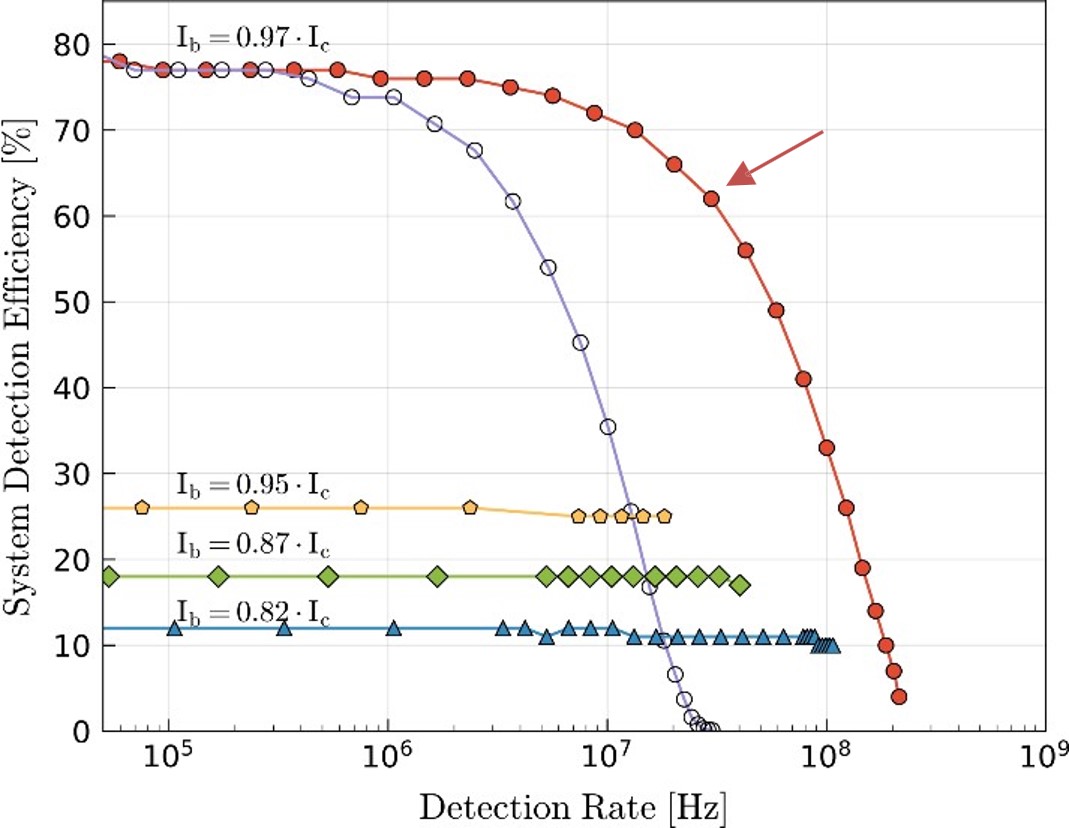
Figure 3: (from Matthieu Perrenoud et al. [1]) Changes in system detection efficiency with the measured detection rate, for regular SNSPDs (lower/left-hand curve, violet) and parallel-pixel SNSPDs (upper/right-hand curve,red).
| The performance of the University of Geneva’s device exceeded expectations. As shown in Figure 3, the low- rate detection efficiency for the parallel SNSPD design was as strong as a regular single-pixel SNSPD, comfortably above 75% efficiency. At high count rates, however, the parallel design comes into its own.
At a detection rate of 10 million photons/s, the regular SNSPDs’ detection efficiency had dropped significantly, and was essentially zero beyond 20 million photons/s. In contrast, the parallel-pixel SNSPDs were still operating at close to peak performance.
Increasing the detection rate further, the parallel SNSPDs can count over 100 million photons/s while still above 30% system efficiency. Impressively, the parallel-pixel detector still counts 200 million photons/s with a system efficiency of 6.6%. |
This order-of-magnitude improvement is a valuable addition to an already high-performance instrument: the ultra-low noise and superb timing precision of current SNSPD technology, with the high count-rates needed for future-proof TCSPC. From the quantum optics lab, to long-haul quantum communication, to high-precision high-sensitivity microscopy, LiDAR and photonic sensing applications, we can’t wait to see how your research will benefit.
To discuss your high-speed single-photon applications with us, or to enquire about the ID281 Superconducting Nanowire Parallel-Pixel model, email us at info@simtrum.com, or visit us at Infrared Single Photon Detector(SPD) or Super Conducting Nanowire System today.
*This article is kindly shared with the permission of ID Quantique for marketing and promotional purposes.